Using 3D petrography of microlites and bubbles in obsidian to assess factors governing eruptive style for rhyolitic magmas
Overview: This four-student advanced Keck project will investigate the factors controlling eruption style in rhyolite volcanic systems, which are among the largest and most destructive on Earth. The project will kick off with a visit to the spectacular Long Valley caldera in central-eastern California to begin building our understanding of rhyolitic systems and to sample young lava domes of the Inyo and Mono craters volcanic chains. Relocating to San Antonio we will then embark on a 3D micro-textural and in-situ analytical study of flow-banded obsidian from Long Valley and from heavily dissected and deeply exposed rhyolite lavas, pyroclastic deposits, and associated vent structures from the largest volcano in eastern Australia. Students will use: (1) bubble and microlite size, shape, number, and orientation distributions to constrain magma ascent and eruption dynamics; and (2) mineral and glass major-element and volatile concentrations to reconstruct pre-eruptive magma storage conditions and to track potential changes in magma properties and compositions during eruption.
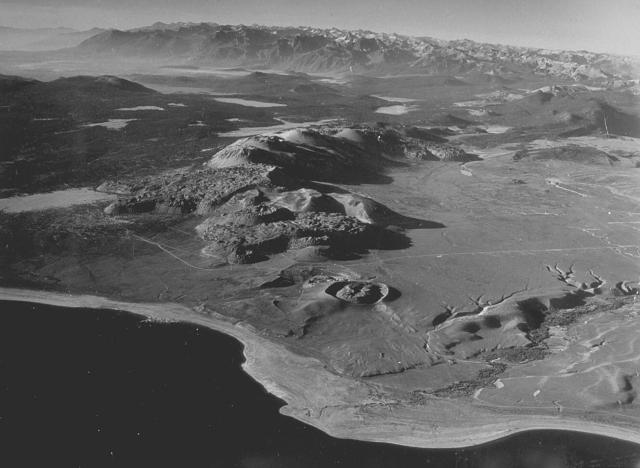
Aerial photo of the lava domes and coulées of the Mono-Inyo craters volcanic chain, looking south from Mono lake towards Long Valley caldera and Sierra Nevada mountains (Photo by R. Von Huene, 1971, United States Geologic Survey)
When: June 15 – July 13, 2019 (tentative)
Where: Long Valley, California (introduction and field work) and San Antonio, Texas (lab work).
Who: Four rising senior students and project leader Dr. Kurt Knesel, Trinity University, kknesel@trinity.edu
Prerequisites and Recommended Courses: Students should have taken an Earth materials course, covering basic mineralogy & petrology. The ideal student would have also had (or would be taking during Fall 2019) a more advanced course in either igneous petrology or structural geology. Some background in chemistry and physics is also recommended but not required. We are particularly interested in applicants with an interest in volcanology, who are comfortable and enthusiastic about working in both outdoor and laboratory environments, and who want to use this work to complete a senior thesis (or equivalent) in geology.
Expectations and Obligations:
1. Participation in field work and data acquisition and analysis during the summer (approximately June 15 to July 13, 2019)
2. Follow-up data acquisition and analysis at home institution and regular correspondence with research team throughout the 2019-2020 academic year.
3. Write an abstract and present a paper (poster or talk) for either the Geological Society of America Cordilleran or Rocky Mountain section meeting in Pasadena, California (May 12-14), or Provo, Utah (May 4-5), respectively.
4. Write a short contribution (4-6 pages text + figures) to be published in the Proceedings of the Keck Geology Consortium 2020 Volume (first draft due Mid-February, 2020).
5. Expected but not required: Use this work for the completion of a senior thesis (or equivalent) at your home institution.
PROJECT DESCRIPTION
Background & Significance
Deciphering the factors governing eruption of intermediate to silicic magma is a fundamental problem in volcanology. A general model has emerged relating eruptive style to the interplay between ascent rate and the efficiency and extent of volatile loss during magma transport from subvolcanic chambers (e.g., Gonnermann & Manga, 2007). Fast ascent is believed to inhibit volatile loss, leading to volatile overpressure and ultimately explosive fragmentation; in contrast, slow magma ascent favors gas escape and lava extrusion. Rise-rate estimates for magmas of andesitic and dacitic compositions are indeed consistent with this model (e.g., Rutherford & Gardner, 2000). However, comparable data for rhyolitic eruptions is sparse (e.g., Costa & Dingwell, 2009; Pallister et al., 2013), and the extent to which ascent rate dictates degassing and eruptive behavior is unclear (e.g., Castro & Gardner, 2008).
Creative approaches to this critical problem are sorely needed. One promising avenue is quantitative 3-D petrographic study of the size, shape and orientation distributions of vesicles and microlites in flow-banded obsidian (i.e., rhyolitic volcanic glass with distinct bands typically defined by differences in abundance of microlites and/or bubbles). For example, orientations of dilute concentrations of microlites can be used to estimate the magnitude and type of shear strain accumulation during magmatic flow (e.g., Manga, 1998; Castro et al., 2002), while bubble shapes and orientations provide constraints on flow stresses and strain rates (e.g., Rust & Manga, 2002; Rust et al., 2003; Rust & Cashman, 2007). When combined, microlite and bubble measurements for the same samples provide a powerful tool for investigating the rates, timescales and dynamics of magma ascent and eruptive emplacement.
We have begun to apply this approach to highly dissected rhyolite domes, pyroclastic deposits, and associated feeder conduits of the compositionally bimodal Tweed volcano, in eastern Australia. Our initial measurements of microlite orientations (Figure 1) within the basal obsidian of a large lava dome yield simple-shear strains of up to 12 (Brown, 2010; Cook, 2011). Relative to an average pure-shear strain of 1 to 2 derived from fold geometries higher in the dome (Smith & Houston, 1994) and strains of < 5 for flow front and upper surface samples of other rhyolite domes (Castro et al., 2002; Befus et al., 2014; Befus et al., 2015), our estimates are remarkably high. Measured shapes and orientations of deformed bubbles (Figure 2) are also consistent with dominantly simple shear in the basal obsidian and allow calculation of shear rates on the order of 10-5s-1 (Knesel et al., 2011). Collectively, these results indicate that the basal portions of lava domes may accommodated most of the deformation during emplacement, while the main mass of lava is rafted above.
Figure 1: Example of 3D microlite reconstruction and strain estimates derived from variance in microlite alignment. (A) Scan of thin section of flow-banded obsidian showing location (yellow star) of area imaged in photomicrograph in (B). (B) photomicrograph of microlites in obsidian (scale bar is ~20 microns). (C) 3D microlite reconstruction derived from a stacked set of digitized images taken from sequential focal depths within the thin section. (D-F) Histograms showing trend direction for three reconstruction locations showing the range of estimated (simple shear) strain derived by comparison of variance in microlite orientation distributions to simulation of theoretical distributions obtained by integration of the relevant equations of motion (e.g., Manga, 1998) for three sample locations.
If microlite and bubble orientations mainly reflect flow at the surface, the average strain and strain rate for individual samples can be used to assess emplacement timescales. Our preliminary data indicate that some large lava domes (>1km3) may be emplaced over a period as brief as a few months, and fed by rhyolitic magma ascending at a rate potentially approaching 1m/s. This ascent estimate is unexpectedly fast and similar to pre-fragmentation rise-rate estimates for explosively erupted rhyolite at Chaitén volcano in Chile (Castro & Dingwell, 2009). If correct, it would appear that, contrary to conventional view, explosive rhyolitic volcanism may not be an inevitable consequence of rapid ascent alone. This possibility requires a re-assessment of current eruption models for rhyolitic systems.
Overarching Goal & Hypotheses
Our overarching goal is to better constrain the factors determining whether rhyolitic magma erupts explosively or effusively. Our starting hypothesis is that magma rise rate dictates the style of rhyolitic volcanism, with fast ascent leading to explosive eruption and slow ascent favoring effusive behavior (e.g., Gonnermann & Manga, 2007). However, as noted above, some of our preliminary results indicate that rapid rise of coherent (pre-fragmentation) rhyolitic magma may not necessarily lead to explosive eruption. An alternative hypothesis is that explosive fragmentation may result from sudden decompression of rhyolitic magma resident in a conduit prior to eruption, rather than simply by a fast-ascent mechanism (e.g., Castro & Gardner, 2008). In this second scenario, some Plinian and Sub-Plinian eruptions may be more akin to Vulcanian eruptions typical of intermediate magma compositions and may ultimately reflect the associated build up and alleviation of exsolved volatile pressure in the conduit transport system (e.g. Castro & Gardner, 2008).
Study Areas
The project includes examination of samples from two volcanic systems: the Mono-Inyo volcanic chain, Long Valley caldera (California), and rhyolite lavas and associated pyroclastic deposits of the Tweed volcano (Australia). The Tweed volcanic center has been the main research site for our structural analysis of flow-related textures over the past decade. The heavy dissection of this Miocene-age system provides us with unprecedented access to the basal glassy portion of rhyolite lava domes, as well as excellent exposure of cogenetic pyroclastic deposits and associated vent structures. In contrast, the Holocene Mono-Inyo chain in California provides easy access to the glassy upper and flow-front portions of rhyolite domes, and, due to its young age, is less susceptible to volatile redistribution following emplacement. Because we already have an extensive obsidian sample set for the Australian system (developed through the course of three honors theses at the University of Queensland), the field portion of the project will target the Mono-Inyo chain in California.
Research Methods
Field work: We will spend our first day or two in the field exploring key locations of fall and ash-flow deposits of the Bishop Tuff, along with key overlook stops of the spectacular Long Valley caldera, to develop an understanding of how field studies of volcanic rocks provide insight into eruptive dynamics. The remainder of our field time will be spent examining, documenting, and sampling macro-scale flow structures (e.g., orientations and dimensions of flow bands and folds) on the surfaces of young lava domes of the Inyo and Mono volcanic chains. The goal of this field work will be to assess the magnitude, type of strain developed, and displacement field in the outer portions of rhyolite lava domes, providing a comparative point to our investigation of the deeper portions of dissected domes in eastern Australia. As we explore the products of both effusive and explosive eruptions in the field, we will begin to formulate ideas for individual projects.
Quantitative and qualitative 3D micro-textural data: Up to this point my students and I have measured microlite and bubble populations using oriented thin sections and standard research-grade microscopes (Brown, 2010; Cook, 2011; Luck, 2012). The transparent nature of obsidian (Figure 1) allows 3D microlite orientations to be constrained using a series of high-magnification digital micrographs taken serially through different focal depths (i.e., focusing down into the thin section) followed by stacking and digitizing of these serial images in a computer-automated drafting (CAD) program (e.g., Manga, 1998). Quantification of bubble populations, which typically display a larger range in sizes compared to microlite populations, requires examination of two thin sections in mutually perpendicular planes (Figure 2) for each sample to enable the measurement of the three radii of deformed bubbles and the angle between the long dimension of bubbles and the flow direction, as defined by flow bands (e.g., Rust et al., 2003).
We will build on our quantitative textural dataset through use of the High-Resolution X-ray Computed Tomography (X-rayCT) facility at the University of Texas (UT) at Austin. The UT X-rayCT lab is a NSF-supported national shared multi-user facility, and is well set up to facilitate post-analysis image processing and visualization of scan data. The majority of our samples will likely be analyzed using the Xradia microXCT scanner, which has a resolution below 1 micrometer, but larger samples will take advantage of the upgraded North Star Imaging (NTS) scanner.
Microlite size distributions and number densities are byproducts of the 3D textural measurements discussed above. These size and density distributions can provide additional constraints on magma degassing and ascent dynamics (e.g., Toramaru et al., 2008; Melnik et al., 2011), but their application requires careful mineral identification. Towards this end, we will begin to quantify microlite populations (feldspar, pyroxene, and Fe-oxides) using the energy-dispersive (EDS) capability of the JOEL JSM-6010LA SEM in the Center for Science and Innovation at Trinity; for students with SEM capabilities at their home institution, this microlite quantification can be continued during the academic year.
Melt and mineral compositional data: Estimates of pre-eruptive storage conditions are critical to assessing degassing during ascent and to estimating melt viscosity. Importantly, the latter is also required to derive strain rates from bubble deformation data. We will determine magma storage conditions (pressure, temperature, volatile content) through analysis of phenocrysts, matrix glass, and quartz-hosted glass inclusions. Major elements will be determined by electron microprobe (EMPA) at UT and/or students’ home institutions. Volatile contents will be measured by Fourier-Transform Infrared (FTIR) spectroscopy at UT and/or students’ home institutions.
Figure 2: Example of the use of bubble orientations in obsidian to estimate shear rates. (A) Photomicrograph oriented perpendicular to the shear plane (containing the shear direction) suitable for measuring the semi-principal axes l, b and the angle between l and the flow direction, as shown in (C). Scale bars in (A & B) are ~200 microns. (B) Photomicrograph in plane perpendicular to bubble lineation used to measure b and c. (D) Fit to b-c bubble axis data measured from orientation in (B) used to calculate c values for data in (A), which allows derivation of the radius for undeformed bubbles using equation in (C). (E) Fit to “small deformation” bubble data (Ca<1) used to derive Ca/a ratio and ultimately to calculate the strain rate from the expression for the capillary number (Ca).
Potential Student Projects: While we envision a wide range of potential projects, our hope is to foster a collaborative research environment where all students feel comfortable sharing and integrating their results and to work as a team to address our overriding goal. As we begin to define each project, on the bases of student interests and consideration of availability of analytical equipment at their home institutions, it will be important to consider the following questions:
• Do microlite (& bubble) alignment reflect flow in the vent, flow on the surface, or both?
• How fast is fast? What is the upper limit of flow velocities (in shallow conduits) separating effusive and explosive eruptions?
• Over what time scales are rhyolite lava domes emplaced?
• How does strain accumulate across lava domes and what role does strain localization play in dome emplacement?
• What is the origin of pyroclastic obsidian?
• How do microlite and bubble textures in obsidian vary between lava, pyroclast, and vent samples?
• To what extent do pre-eruptive magma conditions in the feeder system, as well as storage chambers, play in determining eruptive style?
• How and where does rhyolitic magma degas?
Any one or more of these research questions could form the basis of an individual student research project. Each student could be involved in the acquisition and integration of a range of data types (quantitative textural data, mineral/glass major-element data, glass water and CO2 contents, etc.) for one type of volcanic deposit/structure (i.e., dome vs. pyroclastic vs. vent obsidian). Alternatively, some projects could primarily focus on an individual technique (e.g., FTIR) and data type (e.g., volatile contents) and could include samples from one, two, or all three deposit/structure types. Although we don’t plan to strictly predefine individual projects at the onset of the summer program, five options are listed below to provide a starting point for discussion.
Project 1: Pre-eruptive storage conditions and eruption dynamics of rhyolite lava.
Project 2: Origin of pyroclastic obsidian and implications for pre-fragmentation magma ascent in explosive eruptions.
Project 3: Magma flow dynamics and degassing within an exposed rhyolite vent.
Project 4: Breaking magma: repeated fracturing and healing of melt and the localization of strain in the basal shear zone of a lava dome.
Project 5: Structural analysis of flow foliations and folds as indicators of rheology and 3D kinematics of lava-dome emplacement.
Ultimately, individual projects will be formulated based on both student interests and availability of analytical equipment (e.g., SEM, EMPA, FTIR) at their home institutions. However, all students will gain exposure to cutting-edge high-resolution imaging techniques (SEM & X-rayCT), as well as experience in basic field techniques. All students will learn to:
• Properly document field data, including notebook organization and field-sketching techniques and field-based photography;
• Map and sample structural features of lava domes and flows using standard field equipment;
• Observe and analyze both qualitative and quantitative petrographic information (obtained from traditional optical microscopy) in the context of a focused research project, including microlite and bubble size and orientation data, shear flow indicators (e.g., rotated phenocrysts), folding of flow bands, and brittle-ductile textures (Trinity and home institutions);
• Measure and analyze qualitative and semi-quantitative textural and chemical data using an SEM with EDS (Trinity and home institutions);
• Utilize state-of-the-art X-ray computed tomography (X-rayCT) techniques to obtain and visualize 3D textural data for volcanic samples (UT).
PROJECT LOGISTICS
The summer portion of the project will tentatively run from June 15 to July 13, and will involve a combination of field work (Long Valley, CA) and laboratory work (Trinity and University of Texas).
The first quarter of the project will be dedicated to field work at Long Valley caldera and the associated Inyo-Mono volcanic chain. Students will fly directly to LA, where we will meet at LAX, rent a vehicle, and drive to Bishop (about 5 hours driving time). While in the field we will plan to stay at the Owens Valley Station (OVS) of the White Mountain Research Station (WMRS). The OVS is located about 4 miles east of the town of Bishop at an elevation of 4108 ft; it has air-conditioned dorms and provides excellent cooked meals. We tentatively plan to drive back to LA on morning of June 24th and fly to San Antonio in the evening.
The remainder of the time in the summer will be spent working at Trinity and the University of Texas in Austin. Students will stay in dorms at Trinity with other summer research students. Most dorms have kitchens facilities, so meals can be prepared on campus. Given that our stay at Trinity will coincide with the summer academic session, there should also be access to the main dining facility on campus (Mabee Dining Hall).
Upon arrival at Trinity, we will follow up our field work with a 3-day short course meant to: (1) provide an overview of volcanism in eastern Australia and a hands-on introduction to the associated obsidian sample set, which will complement the samples we collected from the Mono-Inyo chain; (2) an overview of the analytical techniques that we will employ at Trinity, UT, and/or students’ home institutions; and (3) an introduction to the theoretical aspects of magma flow and deformation, including application of quantitative microlite and bubble data. During this stage, we will begin examining the macro and micro-textures of the obsidian samples (in hand-samples and in thin-sections), as we work to refine the individual student projects.
Once the projects are set, students (both individually and collectively) will concentrate on: (1) developing a research proposal, including a timeline for the academic year; (2) sample preparation (e.g., thin/thick-section billet preparation, carbon-coating existing thin-sections, cylinder coring for XrayCT); and (3) initial data acquisition and treatment (primarily SEM work at Trinity & XrayCT work at UT). The close proximity of Trinity and UT (~ 1-hour dive) should allow us to organize sample preparation, data acquisition and treatment, and initial data analysis as needed.
Once at their home institutions, students will continue data collection and analysis as set out in their research proposals. Monthly or, if necessary, bi-weekly Skype, Facetime and/or WhatsApp meetings will be organized with each student and their home advisor (when possible) and with all of the students as a group (when appropriate) to ensure we stay on track to meet our individual and collective goals.
Safety
As with any field and lab work there will be inherent safety concerns. Field hazards include dehydration, heat exhaustion, sunburn, insect bites, poisonous snakes, and physical injuries, such as sprained ankles, broken bones, and skin abrasions or lacerations, as well as potential personal injury due to vehicular accident during travel to and from the field area and daily trips to individual field sites. Lab risks for this project are relatively minor but do include possible skin lacerations or abrasion when cutting or coring samples for preparation of thin/thick section billets and for X-rayCT samples. Risks will be mitigated by adhering to proper safety protocols, including wearing appropriate protective equipment and following standard operating procedures, both in the field and in the lab. We will hold safety meetings where we identify hazards and assess risks, and outline appropriate control measures.
Although summertime high temperatures at the Owens Valley station (at an elevation of just over 4000 ft) are typically in the mid 90s, the majority of field sites in the Mono-Inyo craters area are between 7000 to 8000 ft, where summertime highs are typically 70-80ºF and average lows are in the low to mid 40s. Thus, layered clothing is necessary, and a medium-sized day pack is essential to allow for clothing changes and sufficient food and water (several large bottles) for each day. Sturdy hiking boots and gloves (ideally leather) are also key for safety while working on blocky lava flows and domes.
PROFESSIONAL DEVELOPMENT
Towards the end of the 2019-2020 academic year, we plan to take all participants to either the GSA Cordilleran (May 12-14) or Rocky Mountain (May 4-5) section meeting in Pasadena, CA, or Provo, Utah, respectively, to present their results. We hope that most students will be first author on one paper (poster or talk), and probably secondary authors on others due to the collaborative nature of the project. The project director will work with each student and their home advisor to facilitate this important step in their professional development. And, recognizing that the timing of GSA section meetings may present issues with exam and other class-project deadlines towards the end of the academic year, the project director will coordinate with each student and their home advisor to select the most appropriate meeting.
In addition, all students are required to complete a 4-6 page (short communication) that will be published in the Proceedings of the Keck Geology Consortium 2020 Volume (see examples from previous years). The first draft of this paper will be reviewed by your research advisor at your home institution in February, 2020, with the revised version sent to the project director by March 1. Final versions of your paper and figures will be submitted to the Keck Office in mid-March.
References
Befus, K.S., Zinke, R.W., Jordan, J.S., Manga, M., & Gardner, J.E. (2014) Pre-eruptive storage conditions and eruption dynamics of a small rhyolite dome: Douglas Knob, Yellowstone volcanic field, USA. Bulletin of Volcanology 76, 1-12.
Befus, K.S., Manga, M., Gardner, J.E., & Williams, M. (2015) Ascent and emplacement dynamics of obsidian lavas inferred from microlite textures. Bulletin of Volcanology 77, 1-17.
Brown, A. (2010) Flow dynamics, strain history and structural evolution of the basal shear zone of silicic lava, Minyon Falls Rhyolite, New South Wales, Australia. Unpublished Honors Thesis, University of Queensland, pp. 53.
Castro, J., Manga, M., & Cashman, K. (2002) Dynamics of obsidian flows inferred from microstructures: insights from microlite preferred orientations. Earth and Planetary Science Letters 302, 38-50.
Castro, J.M. & Gardner, J.E. (2008) Did magma ascent rate control the explosive-effusive transition at the Inyo volcanic chain, California? Geology 36, 279-282.
Castro, J.M. & Dingwell, D.B. (2009) Rapid ascent of rhyolite magma at Chaiten volcano, Chile. Nature 461, 780-784.
Cook, 2011 Ascent and emplacement history of silicic lava inferred from microlite and bubble shapes and orientations Minyon Falls Rhyolite, New South Wales, Australia, Unpublished Honors Thesis, University of Queensland, pp. 250.
Gonnermann, H.M. & Manga, M. (2007) The fluid mechanics inside a volcano. Annual Review of Fluid Mechanics 39, 321-356.
Knesel, K., Brown, A., Cook, S. & Luck, D. (2011) Strain history and emplacement dynamics of lava domes: insights into shear-fragmentation and degassing of silicic magma. Biennial Conference of the Geological Society of Australia Specialist Group in Geochemistry, Mineralogy & Petrology, Murramarang, Australia.
Luck, D. (2012) Flow dynamics and eruptive history of silicic magma from the Tweed shield volcano, using bubble deformation analysis. Unpublished Honors Thesis, University of Queensland, pp. 138.
Manga, M. (1998) Orientation distribution of microlites in obsidian. Journal of Volcanology and Geothermal Research 86, 107-115.
Melnik, O.E., Blundy, J.D., Rust, A.C. & Muir, D.D. (2011) Subvolcanic plumbing systems imaged through crystal size distributions. Geology 39, 403-406.
Pallister, J.S., Diefenbach, A.K., Burton, W.C., Munoz, J., Griswold, J.P., Lara, L.E., Lowenstern, J.B., & Valenzuela, C.E. (2013) The Chaiten rhyolite lava dome: Eruption sequence, lava dome volumes, rapid effusion rates and source of the rhyolite magma. Andean Geology 40, 277-294.
Rust, A.C., & Manga, M. (2002) Bubble shapes and orientations in low Re simple shear flow. Journal of Colloid and Interface Science 249, 476-480.
Rust, A.C., Manga, M. & Cashman, K.V. (2003) Determining flow type, shear rate and shear stress in magmas from bubble shapes and orientations. Journal of Volcanology and Geothermal Research 122, 111-132,
Rust, A.C. & Cashman, K.V. (2007) Multiple origins of obsidian pyroclasts and implications for changes in the dynamics of the 1300 B.P. eruption of Newberry Volcano, USA. Bulletin of Volcanology 69, 825-845.
Rutherford, M.J. & Gardner, J.E. (2000) Rates of magma ascent. In Encyclopedia of Volcanoes (Ed. Sigurdsson, H.) 207-217.
Smith, J.V. & Houston, E.C. (1994) Folds produced by gravity spreading of a banded rhyolite lava flow. Journal of Volcanology and Geothermal Research 63, 89-94.
Toramaru, A., Noguchi, S., Oyoshihara, S. & Tsune, A. (2008) MND (microlite number density) water exsolution rate meter. Journal of Volcanology and Geothermal Research 175, 156-167.